Anitra C. Carr1,
Principles of Nutritional
Assessment: Vitamin C
3rd Edition, August 2024
Abstract
Vitamin C (ascorbic acid) is involved in a variety of biological processes, acting primarily as an electron donating enzyme cofactor and reducing agent (antioxidant). Clinical deficiency of vitamin C results in scurvy, a relatively rare disease in high-income countries. Nevertheless, moderate vitamin C deficiency (hypovitaminosis C) has been described in selected vulnerable groups such as the institutionalized elderly, with gingival inflammation and fatigue appearing to be the most sensitive clinical markers for moderate vitamin C deficiency. Excess intakes of vitamin C may produce osmotic diarrhea in some people, and although oxaluria has been reported, this measurement is prone to errors in the preparative or investigative procedure. There are currently no reliable functional indices of vitamin C nutriture. Static biochemical indices such as plasma or leukocyte ascorbic acid concentrations are most frequently used. The former reflect recent dietary intake within a limited range (30–80mg/d). Plasma ascorbic acid concentrations increase linearly with dietary intake but only up to a plateau of about 70µmol/L, and thus are unsuitable for detecting excessive intakes of vitamin C. A number of non-nutritional factors, including sex, body weight, smoking, and severe infection, affect plasma ascorbic acid concentrations. Plasma concentrations are a more reliable marker of vitamin C status than dietary intake assessments. Urinary excretion of vitamin C occurs when the renal reabsorption threshold has been reached and plasma “saturation” occurs (between about 60 and 70µmol/L). Urinary excretion is influenced by recent dietary intake. Moreover, the sensitivity and specificity of this test is low, and 24h urine samples are generally required. Leukocyte ascorbic acid concentrations are considered a relatively reliable index of tissue ascorbic acid stores in healthy people; they are less responsive than plasma to short-term fluctuations in vitamin C intakes, but their assay is difficult and requires a larger blood sample. Furthermore, leukocyte ascorbic acid concentrations may not be a reliable marker of vitamin C status in some conditions, such as severe infection. The body pool size of vitamin C has been estimated using isotope dilution techniques. More research is needed to identify useful functional tests of vitamin C status. CITE AS: Anitra C. Carr, Principles of Nutritional Assessment: Vitamin C https://nutritionalassessment.org/vitaminc/Email: anitra.carr@otago.ac.nz
Licensed under CC-BY-4.0
( PDF )
19.1 Vitamin C
For centuries, the consumption of citrus fruit has been associated with the prevention of the vitamin C deficiency disease scurvy, but it was not until 1932 that the active compound was isolated (King & Waugh, 1932; Svirbely & Szent-Györgyi, 1932). A year later, ascorbic acid was characterized and became the first vitamin to be chemically synthesized. Humans are unable to synthesize vitamin C in vivo from glucose due to random genetic mutations, which occurred at an early stage in our evolution, and have resulted in loss of the terminal enzyme in the biosynthetic pathway, L‑gulonolactone oxidase (Nishikimi & Yagi, 1991; Drouin et al., 2011).
The term “vitamin C” is used here to
embrace both L‑ascorbic acid and its
oxidised form, L‑dehydroascorbic acid; both have
approximately equal vitamin C activity as dehydroascorbic
acid can be readily reduced back to the biologically active
ascorbic acid in the body. Ascorbic acid is the enolic form
of an α‑ketolactone
(2,3-didehydro-threo-hexano-1,4-lactone), which in
solution can be oxidized to the diketo form—
dehydro-L‑ascorbic acid; their structures are shown in
Figure 19.1.
19.2 Functions of Vitamin C
Ascorbic acid acts as an electron donating cofactor for a family of biosynthetic and regulatory metalloenzymes that carry out important hydroxylation reactions (Englard & Seifter, 1986; Kuiper & Vissers, 2014; Young et al., 2015). These include copper-containing mono-oxygenases and iron-containing dioxygenases. Of the latter, the most well-known is hydroxylation of proline and lysine residues during synthesis of collagen, the major connective tissue protein in the body. Ascorbic acid acts in these hydroxylation reactions by reducing the metal catalytic sites of procollagen proline 4‑dioxygenase (EC 1.14.11.2) and procollagen lysine 5‑dioxygenase (EC 1.14.11.4). Decreased activity of these two enzymes can lead to the defects in connective tissue that in turn cause the characteristic clinical features of scurvy. These include joint pain, tooth loss, bone and connective tissue disorders, and poor wound healing. Ascorbic acid is also involved in the hydroxylation of dopamine to noradrenaline, by acting as a cofactor for the enzyme dopamine-β-monooxygenase (EC 1.14.17.1). Noradrenaline is a neurotransmitter, and deficient dopamine hydroxylation may be associated with the mood changes, depression, and hypochondria that can occur with scurvy. The early symptoms of scurvy — fatigue, lethargy, and muscle weakness — are related to the role of ascorbic acid as a cofactor for maximal activity of two dioxygenases involved in carnitine biosynthesis (N‑trimethyllysine dioxygenase; EC 1.14.11.8, and γ‑butyrobetaine dioxygenase; EC 1.14.11.1). Carnitine is essential for the transport of activated long-chain fatty acids into the mitochondria, where they are converted to energy by way of β‑oxidation (Englard & Seifter, 1986). Several other enzymes depend on ascorbic acid as a cofactor, although their specific role in the development of scurvy is still unclear. These include peptidylglycine α‑amidating monooxygenase (EC 1.14.17.3), an enzyme required for the synthesis of numerous amidated peptide hormones which have multiple functions throughout the body (Englard & Seifter, 1986). More recent discoveries have included the hydroxylases that regulate the concentration and activity of the transcription factor hypoxia-inducible factor‑1α (i.e. hypoxia-inducible factor — proline dioxygenase; EC 1.14.11.29, and hypoxia-inducible factor — asparagine dioxygenase; EC 1.14.11.30) (Kuiper & Vissers, 2014), and the ten-eleven translocation (TET) hydroxylases and histone demethylases that modify epigenetic marks on DNA and histones (Young et al., 2015). Together, these epigenetic and transcription regulating enzymes have the potential to up- and down-regulate thousands of genes in the body, likely contributing to vitamin C's pleiotropic functions in human health and disease. Ascorbic acid also has several nonenzymatic functions based on its role as a reducing agent and antioxidant (i.e. electron donation). For example, ascorbic acid enhances the gastrointestinal absorption of nonheme iron, through its ability to reduce intraluminal iron to its more absorbable ferrous form (Hallberg et al., 1987). Ascorbic acid is a potent antioxidant, with the ability to quench a wide range of reactive oxygen species, including neutralising phagocyte-derived oxidants, thus attenuating tissue damage (Carr & Frei, 1999a). Ascorbic acid provides important antioxidant protection to plasma lipids and lipid membranes through direct scavenging of reactive oxygen species and through the regeneration of vitamin E from its oxidised form (α‑tocopheroxyl radical). Ascorbic acid can also regenerate the enzyme cofactor tetrahydrobiopterin from its oxidised form (dihydrobiopterin), thus potentially aiding synthesis of dopamine, serotonin and nitric oxide. At high intakes, vitamin C has been associated with protection against cardiovascular disease, cataracts, and cancer (Carr & Frei, 1999b), and a variety of immune-related functions (Carr & Maggini, 2017). Additional research, however, is needed to establish causal relationships between vitamin C and these degenerative diseases and immunocompetence.19.3 Absorption and metabolism of vitamin C
Vitamin C is absorbed in the small intestine by an active sodium-dependent vitamin C transporter (SVCT‑1) that is dose dependent and can become saturated (Savini et al., 2008). About 70%–90% of vitamin C is absorbed when daily intakes range from 30 to 180mg, but absorption falls to ≤ 50% at intakes above 1g/d (Kallner et al., 1979; Levine et al., 1996). Indeed, the osmotic diarrhea and intestinal discomfort sometimes experienced by persons ingesting large gram doses of vitamin C is due to the presence of a large amount of unabsorbed vitamin C. Regulation of the whole body vitamin C content is achieved in part by this dose-dependent intestinal absorption of vitamin C. Renal action to conserve vitamin C (via SVCT‑1) or excrete unmetabolized vitamin C also plays an important part. Tissue uptake occurs primarily by the SVCT‑2 isoform (Savini et al., 2008). Different tissues uptake and retain vitamin C to variable extents, with neuronal and glandular tissues containing the highest concentrations (Hornig, 1975), indicating vital roles for vitamin C in these tissues. Catabolism of ascorbic acid in humans occurs through oxidation to dehydroascorbic acid and, in the absence of sufficient reducing equivalents, subsequent hydrolysis to diketogulonic acid. This can then be oxidised to smaller molecules, such as oxalate, that are excreted in urine. However, it should be noted that dehydroascorbic acid is readily taken up via cell membrane glucose transporters (GLUTs) and rapidly reduced back to ascorbic acid via various chemical and enzymatic pathways (Rumsey et al., 1997; Washko et al., 1993), thus limiting dehydroascorbic acid concentrations in vivo. With large intakes, most of the vitamin C is excreted in its unmetabolized form (Levine et al., 1996).19.4 Deficiency of vitamin C in humans
Clinical manifestations of scurvy, such as weakness, petechial hemorrhages, gingival bleeding, ecchymoses and subperiosteal hemorrhage, anemia, and defects in bone development in children, are relatively rare in high-income countries. Nevertheless, vitamin C deficiency has been observed in institutionalized elderly subjects, likely arising from inadequate intake of vitamin C (Carr & Rowe, 2020). Alcoholic patients, those on restrictive diets and people in refugee camps are also vulnerable to vitamin C deficiency. Infantile scurvy is rare because breast milk provides adequate amounts of ascorbic acid and infant formulas are fortified with sufficient ascorbic acid to prevent the disease. Subclinical vitamin C deficiency may develop secondarily to some disease states such as severe respiratory infections, cancer, gastrointestinal disorders, cardiometabolic disorders and inflammatory diseases. This is likely partly due to the enhanced oxidative stress and inflammation associated with many of these diseases, as well as the use of certain drugs (e.g. aspirin) or chemotherapeutic agents that appear to affect the bioavailability or metabolism of ascorbic acid (Basu, 1982; Carr & Cook, 2018). The functional health consequences of both mild and moderate vitamin C deficiency are not well characterized. Gingival inflammation, fatigue, and irritability have been reported in moderate vitamin C deficiency induced experimentally (Leggot et al., 1986; Levine et al., 1996).19.5 Food sources and dietary intakes of vitamin C
Vitamin C is synthesised in plants. However, the vitamin C content of different foods is highly variable (Carr & Rowe, 2020). Values can also depend on the growing conditions, season of the year, stage of maturity, location, and storage time prior to consumption. Exposure to high temperatures, oxidation, or cooking in large amounts of water all reduce the vitamin C content of foods. Major food sources of vitamin C in the diets of most high-income countries are fresh fruits and fruit juices and some fresh vegetables; they can contribute up to 90% of the vitamin C intake. Citrus and kiwifruit are particularly rich sources of vitamin C. Of the vegetables, potatoes can be one of the most important sources of vitamin C in the diets of adults, for example in the U.K. (Gregory et al.,1990). Most staple foods, e.g. rice, wheat, maize and other grains, contain negligible amounts of vitamin C and meat, fish, eggs, and dairy products are also poor sources (Carr & Rowe, 2020). In low-income countries, the supply of food sources of vitamin C is often seasonal, so intakes may vary widely. In The Gambia, for example, intakes ranged from zero to 115mg/d in one community, depending on the seasonal availability of mangos, oranges, and grapefruit (Bates et al., 1994). In humans, the bioavailability of vitamin C from food sources is comparable to that from supplements and is not affected by the type of food consumed (Carr & Vissers, 2013).19.6 Nutrient reference values for vitamin C
Several expert groups including the Institute of Medicine (IOM) in the U.S. and the European Food Safety Authority (EFSA) have derived values for Average Requirements (ARs) and Recommended Intakes (RIs) for vitamin C. The AR (U.S. equivalent, Estimated Average Requirement) is set for a particular group of individuals and is used to assess the prevalence of adequate intakes of vitamin C within that group. The RIs (U.S. equivalent, Recommended Daily Allowance (RDA); EFSA equivalent, Population Reference Intake) are derived from the ARs and its distribution and are used to assess intakes and plan diets for individuals. Recommendations for vitamin C intakes vary greatly around the world, with recommended intakes (RIs) for individuals ranging from as low as 40mg/d in the U.K. to 110mg/d by EFSA (2017). In the U.S., the RDAs established by the IOM in 2000 are 75mg/d and 90mg/d for women and men, respectively (Levine et al., 1996; IOM, 2000). The large discrepancies in the RIs have resulted in calls for harmonization (Carr & Lykkesfeldt, 2020). Higher vitamin C intakes are recommended by all expert groups for pregnant and lactating women due to the needs of the developing fetus and the transfer of vitamin C to breast milk. Furthermore, some countries (e.g., the U.S.) recommend higher intakes for smokers due an enhanced oxidative burden and resultant increase in turnover of vitamin C. Consequently, RIs for smokers may be increased by +20mg/d to +80mg/d, depending on the country. Upper levels of intake (ULs) have also been derived for vitamin C by some expert groups. The ULs represent daily intakes that if consumed chronically over time will have a very low risk of causing adverse effects. Intakes from all sources of vitamin C are considered: food and nutrient supplements. Because vitamin C is water soluble, however, any excess not required by the body is readily excreted, therefore, there is no known upper toxic concentration. Nevertheless, some countries have set ULs ranging from 1–2g/d for adults based on potential gastrointestinal disturbances at very large (> 4g/d) doses. For more details on the application of these nutrient reference values, see Chapter 8b. In addition to diarrhea and other gastrointestinal disturbances, possible adverse effects of excessive intakes of vitamin C include an increase in the absorption of dietary iron potentially leading to iron overload in people with hemochromatosis, and hemolytic anaemia in people with glucose‑6-phosphate dehydrogenase deficiency due to an inability to attenuate hydrogen peroxide generation. Current evidence is insufficient to implicate high intakes of vitamin C as a risk factor in oxalate stone formation. Nevertheless, individuals with hemochromatosis, glucose‑6-phosphate dehydrogenase deficiency, and renal disorders, may be susceptible to adverse effects from excessive intakes of vitamin C (IOM, 2000).19.7 Indices of vitamin C status
There are no reliable functional tests of vitamin C status. Instead, static biochemical tests, particularly the measurement of plasma or leukocyte ascorbic acid concentrations, are most frequently used to assess vitamin C status. These static biochemical tests are discussed in detail below.19.8 Plasma ascorbic acid
Ascorbic acid is transported in the plasma but is not bound to any protein; almost all is present as the ascorbate monoanion. Plasma (or serum) ascorbic acid concentrations are the most frequently used and practical index of vitamin C status in studies of individuals and populations. Concentrations are influenced by recent intake of the vitamin, making fasting blood samples essential.19.8.1 Ascorbic acid pharmacokinetics
Plasma ascorbic acid concentrations exhibit a characteristic sigmoidal relationship with intake, as shown in Figure 19.2. In adults, the steepest change is evident at intakes between about 30 and 90mg/d of vitamin C. When intakes are below about 20mg/d, most of the vitamin C enters the tissues, so there is very little available for circulation in the blood. For intakes greater than 100mg/d, plasma concentrations tend to plateau between 60 and 70µmol/L, concentrations representing the renal threshold and plasma saturation (Figure 19.2).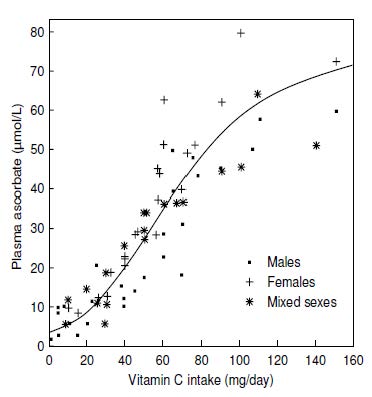
19.8.2 Factors affecting plasma ascorbic acid
Cigarette smoking has a marked effect on plasma ascorbic acid concentrations. Many studies report lower plasma ascorbic acid concentrations in smokers (Carr & Rowe, 2020), as well as in those regularly exposed to environmental cigarette smoke (Figure 19.3).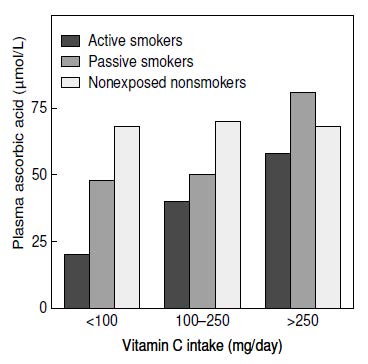
19.8.3 Interpretive criteria for plasma ascorbic acid
Protracted intakes of vitamin C < 20mg/d cause plasma ascorbic acid concentrations to decline rapidly to deficient concentrations (i.e. 11µmol/L or less) (Hodges et al., 1971; Jacob et al., 1987). At the lower plasma ascorbic acid concentrations, clinical signs of scurvy such as follicular hyperkeratosis, swollen or bleeding gums, petechial hemorrhages, and joint pain occur (Hodges et al., 1969). Therefore, plasma ascorbic acid concentrations ≤ 11µmol/L are taken as indicative of vitamin C deficiency. The cut-off values for plasma ascorbic acid concentrations used to distinguish different categories of deficiency are poorly defined. NHANES II defined “clinically based categories” of plasma ascorbate concentrations in terms of mg/dL, i.e. deficient < 0.2, low 0.2–0.39, normal 0.4–0.99, and saturated ≥ 1.0mg/dL (equating to < 11, 11–22, 23–56, and ≥ 57µmol/L, respectively) (Loria et al., 1998). Of note, the low (hypovitaminosis C) cut-off is when the “sub-clinical” signs and symptoms of vitamin C deficiency start to be observed, e.g. changes in mood and energy levels. More recently, the European Food Safety Authority (EFSA) has defined ≥ 50µmol/L as “adequate” (Table 19.1; EFSA, 2013). Well-conducted pharmacokinetic studies in men and women suggest 70µmol/L as a cut-off for “saturation”, although there is variation between individuals (Levine et al., 1996; Levine et al., 2001). The criteria in Table 19.1 have been used in more recent NHANES analyses (Crook et al., 2021), that indicated that smoking, male sex and higher body weight/BMI were common indicators for lower vitamin C status.Status | Plasma Ascorbic Acid (µmol/L) |
---|---|
Deficient | < 11 |
Hypovitaminosis C | < 23 |
Inadequate | < 50 |
Adequate | ≥ 50 |
Saturating | ≥ 70 |
19.8.4 Measurement of plasma ascorbic acid
Fasting blood samples are required for plasma ascorbic acid analysis. The samples need to be preserved immediately after collection to avoid degradation of the ascorbic acid. Metaphosphoric acid (or trichloroacetic acid) is typically used to precipitate protein and to stabilize the ascorbic acid in the samples prior to analysis. Additionally, a reducing agent, such as dithiothreitol (DTT), can be added to preserve the ascorbic acid in a reduced state. The reducing agent TCEP (tris(2-carboxyethyl)phosphine) is versatile in that it can also work in acidified solutions with longer incubation times. A metal chelator, such as DTPA (diethylenetriaminepentaacetic acid), can be added to attenuate metal ion-dependent oxidation of ascorbic acid (particularly important if samples are hemolysed). It should be noted that EDTA (ethylenediaminetetraacetic acid), although a commonly used metal-chelator, is redox-active and can catalyse the oxidation of ascorbic acid if the samples are not handled appropriately (Pullar et al., 2018). Plasma samples for ascorbic acid analysis that have been prepared appropriately and frozen at −20°C are stable for several weeks and at −70°C for at least 1y (Margolis & Duewer, 1996). If the samples cannot be processed immediately, whole blood can be stored for up to 8h at 4°C before processing (Galan et al., 1988). Care needs to be taken with choice of anti-coagulants for collection of blood samples as the anti-coagulant EDTA, although a metal-chelator, remains redox active and can result in loss of ascorbic acid if the blood or plasma samples are not kept at 4°C (Pullar et al., 2018). Several methods are available for measuring vitamin C in both the reduced form or as total ascorbic acid. The older colourimetric or fluorometric assays use predominantly 2,4‑dinitrophenylhydrazine or o‑phenylenediamine. These methods, however, have a number of limitations (Washko et al., 1992). Their sensitivity and specificity is low, and they are often subject to interference by other biological substances and yield falsely high readings at low ascorbic acid concentrations. In some circumstances, inadvertent oxidation of ascorbic acid or hydrolysis of dehydroascorbic acid may occur. The method of choice for measuring ascorbic acid in plasma samples is high-performance liquid chromatography (HPLC); only 50µL of plasma is required for the measurement. Of the HPLC methods available, electrochemical detection is preferred, with its high selectivity and sensitivity. However, this method does require a dedicated instrument and an experienced operator (Washko et al., 1989). Other simpler methods use HPLC coupled with an ultraviolet detector, but these methods can have a lower sensitivity and specificity (Washko et al., 1992). Analysis of dehydroascorbic acid by HPLC is more difficult because direct electrochemical or UV detection of dehydroascorbic acid is not possible. Instead, samples must first be analysed for ascorbic acid and then reduced for measurement of ascorbate plus dehydroascorbic acid. Dehydroascorbic acid is then determined by subtraction. In vivo concentrations of dehydroascorbic acid are generally very low (e.g. < 2µmol/L) due to rapid uptake (via GLUTs) and intracellular reduction to ascorbic acid by cells of the vasculature. Therefore, reports of higher plasma (or serum) concentrations of dehydroascorbic acid in different disease states are likely due to artefactual ex vivo oxidation often associated with use of the older spectrophotometric methods and/or inappropriate handling and processing of the samples prior to analysis (Pullar et al., 2018).19.9 Ascorbic acid in leukocytes and specific cell subsets
Leucocytes include lymphocytes, monocytes, and three classes of granulocytes: polymorphonuclear leukocytes or neutrophils, eosinophils, and basophils. These cell types differ in their concentration of ascorbic acid and possibly in their response to supplemental vitamin C (Levine et al., 1996; Levine et al., 2001), thus complicating the interpretation of ascorbic acid concentrations in leukocytes.19.9.1 Leukocyte ascorbic acid
Concentrations of ascorbic acid in plasma and leukocytes correlate relatively well (Figure 19.4;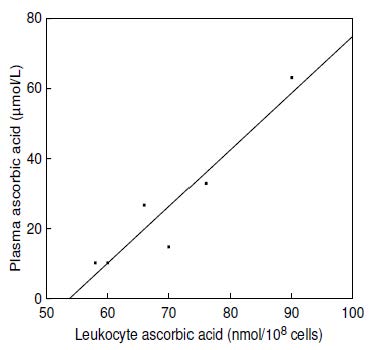
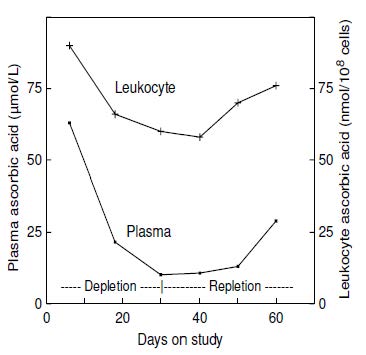
19.9.2 Ascorbic acid in specific cell subsets
The specific cell type that is most useful for assessing vitamin C status is presently uncertain. As noted earlier, both ascorbic acid concentrations in individual leukocytes and their response to vitamin C supplementation vary. Mononuclear cells (lymphocytes and monocytes) have concentrations that are two- to threefold higher than granulocytes (e.g., neutrophils). Furthermore, the ascorbic acid pool in mononuclear cells is depleted more slowly than that from other blood compartments (Jacob, 1990). In addition, it can be difficult to obtain homogeneous and reproducible fractions of specific cell types, so that their reported ascorbic acid concentrations can vary widely. Lymphocyte ascorbic acid concentrations of 120–250nmol/108 cells have been reported for healthy adults not consuming supplemental vitamin C (Evans et al., 1982). Jacob et al. (1991) measured lymphocyte ascorbic acid concentrations in a depletion-repletion study of healthy men that was designed to induce moderate vitamin C deficiency. The mean baseline lymphocyte ascorbic acid concentration in the men was 209nmol/108 cells. After 60d of depletion, lymphocyte ascorbic acid concentrations fell significantly and consistently to a mean concentration of 87nmol/108 cells. Moreover, the concentrations distinguished between the group of participants receiving 5, 10, or 20mg ascorbic acid (74–145 nmol/108 cells) versus those participants receiving 60 or 250mg/d (182–261nmol/108 cells). Strong correlations of plasma and lymphocyte ascorbic acid concentrations were noted within the individual subjects when ascorbic acid intakes ranged from 5–250mg/d (Table 19.2) There was no evidence of scorbutic symptoms in the depletion phase of this study, but there was evidence of greater oxidative damage, based on alterations in indices of oxidant status (Jacob et al., 1991).Days | Ascorbic acid intake (mg/d) | n | Plasma (µmol/L) |
Mononuclear leukocyte (nmol/108 cells) |
---|---|---|---|---|
4 | 250† | 8 | 59.6 ± 3.3a | 209 ± 9a |
32 | 5 | 8 | 6.6 ± 0.3b | 117 ± 7‡b |
28 | 10 | 4 | 5.3 ± 0.3b | 87 ± 9b |
28 | 20 | 4 | 5.9 ± 0.5b | 95 ± 5c |
28 | 60 | 3 | 26.7 ± 8.8c | 201 ± 2a |
28 | 250 | 5 | 64.2 ± 4.5a | 217 ± 11a |
19.9.3 Interpretive criteria for leukocytes
Confusion exists over the interpretive criteria used for leukocyte ascorbic acid concentrations, in part because they can be expressed in different ways: per 108 cells, per mL, per DNA concentration, or per unit of protein. This makes comparisons among laboratories difficult. The most common method is in terms of cell numbers (nmol/108 cells). The heterogeneous nature of the leukocytes and various technical difficulties with their analyses, are further complicating factors. Table 19.3 presents the interpretive criteria used by Jacob (1994) and given in Sauberlich (1999), expressed in terms of cell numbers. When expressed in this way, clinical signs of scurvy, such as swollen or bleeding gums or petechial hemorrhages, have been associated with leukocyte concentrations of about 11nmol/108 cells. Even at concentrations as high as 50nmol/108 cells, scorbutic changes including inflammation, tenderness, bleeding of the gums, and petechiae have sometimes been described (Sauberlich et al., 1989). For this reason, the cut-off shown in Table 19.3, indicative of deficiency, is < 57nmol/108 cells.Status | Mixed leukocytes (nmol/108 cells) | Mononuclear leukocytes (nmol/108 cells) |
---|---|---|
Deficient | < 57 | < 114 |
Low | 57–114 | 114–142 |
Adequate | > 114 | > 142 |
19.9.4 Measurement of leukocyte ascorbic acid
The isolation and assay of leukocytes for ascorbic acid is technically difficult, and presently requires relatively large blood samples (2–5mL), making the assay unsuitable for serial measurements on infants. Leukocytes can be isolated with density-gradient sedimentation (Boyum, 1968). Monoclonal antibodies to various cell types can also be used (Field, 1996). Analysis of ascorbic acid in leukocytes or specific cell types is best performed by HPLC with electrochemical detection, after deproteinization (Washko et al., 1989).19.10 Ascorbic acid in erythrocytes and whole blood
Erythrocyte ascorbic acid concentrations are not widely used as an index of ascorbic acid status. Concentrations are roughly comparable to plasma but only respond to changes in vitamin C intake over a narrow range. Hence, they are not as sensitive as plasma ascorbic acid concentrations (Hodges et al., 1971). Because erythrocyte concentrations are less responsive to recent dietary intake, they could potentially be used to assess ascorbic acid status in non-fasting individuals (Pullar et al., 2020). However, both within- and between-variation and analytical variance can be greater than for plasma ascorbic acid (Jacob et al., 1987). Erythrocyte ascorbic acid is technically more challenging to measure due to potential for oxidation of ascorbic acid by hemoglobin-associated iron; the hemoglobin is ideally removed via centrifugal filter units (Li et al., 2012). It should also be noted that conditions of vitamin C deficiency can result in enhanced erythrocyte fragility and hemolysis (Tu et al., 2015). Whole blood ascorbic acid concentrations have also been investigated in ascorbic acid depletion-repletion studies. Again, concentrations in whole blood are not as sensitive as plasma for assessing ascorbic acid depletion (Jacob et al., 1987). Concentrations < 17µmol/L are considered deficient because they have been associated with scorbutic signs (Sauberlich et al., 1989); those of 17–28µmol/L are considered low. Concentrations > 28µmol/L are interpreted as acceptable (Sauberlich, 1999). Presently, whole blood ascorbic acid concentrations are rarely used.19.11 Urinary excretion of ascorbic acid and metabolites
Urine is the major excretory route for absorbed ascorbic acid. Most ascorbic acid is excreted in its unmetabolized form (Levine et al., 1996). When intakes are > 1g/d, there is some increase in oxalate excretion (Olson & Hodges, 1987). However, reports of high oxalate excretion are likely due to artefactual ex vivo oxidation of excreted ascorbic acid prior to analysis (Chalmers et al., 1985), therefore careful handling of urine samples is required. Urinary excretion of ascorbic acid reflects recent dietary intake. Experimental depletion-repletion studies have demonstrated that concentrations in the urine decline progressively with increasing depletion of vitamin C until, in persons with scurvy, concentrations are undetectable (Jacob et al., 1987). Nevertheless, urinary excretion is not a very sensitive index of ascorbic acid status; differences between persons with adequate or deficient intakes of ascorbic acid are small. For example, in the depletion-repletion study of 11 young men shown in Figure 19.6,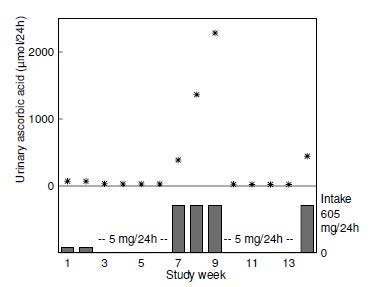