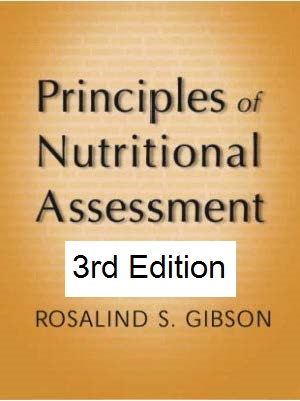
Whiting SJ, Gibson RS.
Principles of Nutritional
Assessment: Vitamin D
3rd Edition August 2024
Abstract
Vitamin D is a generic term for a group of related substances, including vitamin D2 (ergocalciferol) and vitamin D3 (cholecalciferol). It is a precursor to the steroid hormone 1,25‑dihydroxyvitamin D (calcitriol) that helps the body absorb and retain calcium and phosphorus, thus contributing to bone health. Deficiencies in vitamin D may cause rickets, osteomalacia and osteoporosis. Sun-mediated synthesis of vitamin D in the skin may be important in lower latitudes, but in settings where this synthesis is not significant, vitamin D is an essential nutrient. As vitamin D is a fat-soluble vitamin, deficiencies may be caused by malabsorption syndromes. Exposure and status of vitamin D is assessed by measuring the concentration of the transport metabolite, 25‑hydroxyvitamin D, that reflects both ingested and skin-synthesized sources. 1,25‑dihydroxyvitamin D (calcitriol) is not a measure of vitamin D status as levels in blood reflect temporary stimulation and not nutrient stores. Other measures of vitamin D status include factors and molecules related to its role in bone development; little is known about specific biomarkers of the nonskeletal roles of vitamin D
CITE AS:
Whiting SJ & Gibson RS. Principles of Nutritional Assessment:
Vitamin D . https://NutritionalAssessment.org/ vitaminD/
Email: Susan Whiting (sjw084@mail.usask.ca)
Licensed under CC-BY-4.0
( PDF )
18b Vitamin D
Vitamin D (calciferol) is a generic term for a group of related fat-soluble substances, including vitamin D2 (ergocalciferol), vitamin D3 (cholecalciferol) and their metabolites (Figure 18b.1).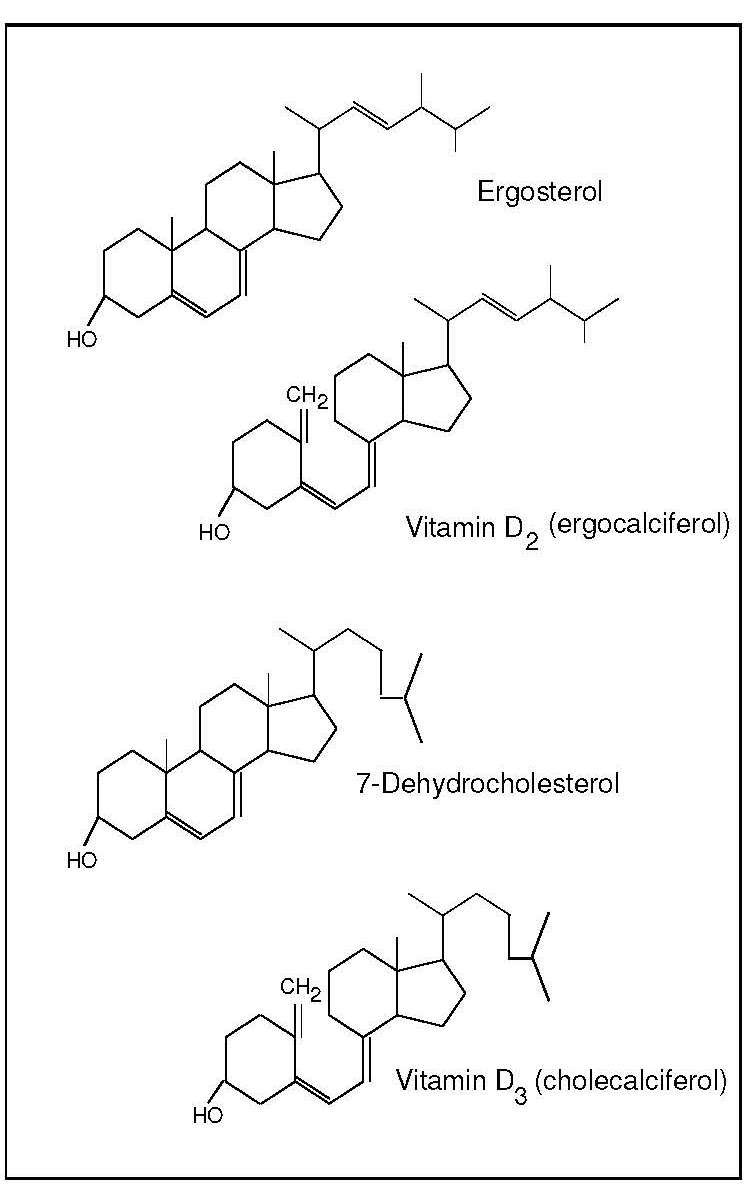
18b.1 Functions of vitamin D
The active form of vitamin D, 1,25‑dihydroxyvitamin D (calcitriol), primarily functions as a steroid hormone, ensuring adequate intestinal absorption of calcium and phosphorus and regulating bone mineralization. In this role, the pathway of synthesis of the active form 1,25‑dihydroxyvitamin D (calcitriol), is endocrine as it is made in a tissue only when it is required, then circulates to target tissues, where it is present for only a short period of time.
Vitamin D also has a critical role in a number of cellular functions in many tissues in the body, including bone, placenta, prostate, keratinocytes, macrophages, T-lymphocytes, dendritic cells, parathyroid gland and some cancers (Wacker and Holick, 2013). The active metabolite 1,25‑dihydroxyvitamin D (calcitriol) is involved in modulating the immune response and in regulating cell differentiation, proliferation, and apoptosis (Norman, 2008). In these non-skeletal roles, the pathway of synthesis of this active form is autocrine / paracrine, whereby the active form stays in the cell or is transported locally.
18b.2 Metabolism of vitamin D
Vitamin D can be obtained in two ways: from skin synthesis of vitamin D3 (cholecalciferol) and from ingestion of the parent compounds (D2 (ergocalciferol) or D3) from foods or supplements. This situation creates problems in assessing vitamin D status as dietary intake alone is not sufficient to gauge risk for deficiency. Sun exposure along with behavioural and environmental factors affecting skin synthesis must also be taken into account.18b.2.1 Skin synthesis of vitamin D3 (cholecalciferol)
The requirement for vitamin D can be met by skin synthesis alone provided UVB can reach the skin. The synthesis of vitamin D3 in the skin involves two stages: the photochemical transformation of 7-dehydrocholesterol to previtamin D3 by UVB, followed by thermal isomerization of the previtamin to vitamin D3 (cholecalciferol). Variables influencing the formation of previtamin D3 in the skin include skin pigmentation, the intensity of the solar ultraviolet light, and environmental factors such as clouds, smog, clothing and sunscreen use (Grant et al., 2016; Wacker and Holick, 2013). Over-exposure to UVB will not lead to excess skin synthesis of vitamin D, as previtamin D as well as vitamin D3 are irreversibly converted to inactive metabolites (Wacker and Holick, 2013). In the absence of UVB exposure, the requirement for vitamin D must be met from dietary sources. Mostly, the requirement is met partially or fully by diet, even in equatorial regions because people may embrace an urban or sun-avoiding lifestyle.
18b.2.2 Vitamin D2 (ergocalciferol)
Vitamin D2 (ergocalciferol) cannot be made by animals. The only source is ingestion of certain foods in the fungi kingdom. It is important to note the fungi kingdom is separate from the other eukaryotic life kingdoms of plants and animals and texts may erroneously refer to vitamin D2 as a “plant” source. Sun-exposed mushrooms naturally provide vitamin D2, while UV-exposed yeast and mushrooms added to the food supply enhance the vitamin D content of foods (Wacker and Holick, 2013).The major metabolic steps involved in the metabolism of vitamin D2 are similar to those for the metabolism of vitamin D3 (cholecalciferol). The evidence about efficacy of vitamin D2 versus vitamin D3 suggests that although vitamin D3 is the more active (Logan et al., 2013), vitamin D2 is a reasonable alternative (Wacker and Holick, 2013). Hence, in the following discussion and in figure 18b.2, the term “vitamin ” refers to either or both vitamin D2 and vitamin D3 and their metabolites.
18b.2.3 Production of 25‑hydroxyvitamin D
Vitamin D enters the circulation from the skin or from the lymph via the thoracic duct, bound to a specific vitamin D-binding protein. Vitamin D is transported to adipose tissue where it is stored, or to the liver, where it is hydroxylated to 25‑hydroxyvitamin D (25(OH)2D [also called calcidiol when referring to 25(OH)2D3], the major circulating form of vitamin D (Figure 18b.2).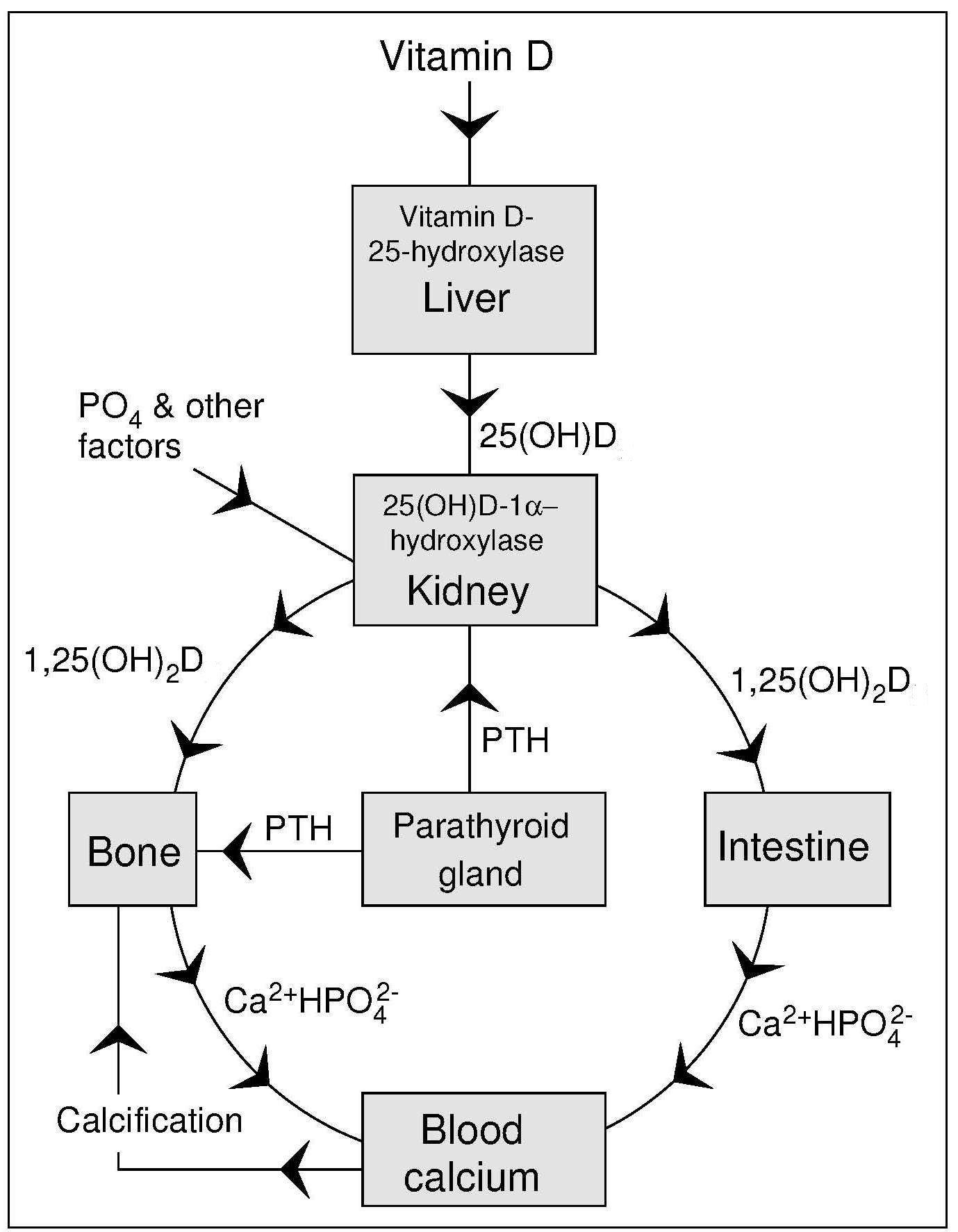
18b.2.4 Renal production of circulating 1,25‑dihydroxyvitamin D (calcitriol)
The active form of vitamin D, 1,25(OH)2D (calcitriol) that circulates in plasma is made in the kidney in the Endocrine Pathway as shown in Figure 18b.2. This synthesis of 1,25(OH)2D is homeostatically controlled, mainly by the action of parathyroid hormone (PTH) in response to serum calcium levels, and fibroblast growth factor 23 (FGF-23) related to serum phosphate levels (Wacker and Holick, 2013), that regulate the activity of renal (25(OH)D-1-α-hydroxylase). For example, a decrease in plasma calcium prompts an increase in parathyroid hormone secretion from the parathyroid gland that acts to mobilize calcium stores from the bone. Parathyroid hormone also promotes the synthesis of 1,25(OH)2D in the kidney which, in turn, stimulates the mobilization of calcium from the bone and increased intestinal calcium absorption (Figure 18b.2). Once plasma calcium levels are normal, the need for circulating 1,25(OH)2D diminishes and there is no stimulation of the enzyme 25(OH)D-1-α-hydroxylase. Thus, circulating levels of 1,25(OH)2D are not related to vitamin D status as in deficiency low levels of 1,25(OH)2D may reflect lack of the precursor metabolite 25(OH)D. However, as vitamin D deficiency leads to secondary hyperparathyroidism with PTH-enhanced 1,25(OH)2D production, vitamin D deficiency can be associated with normal to high 1,25(OH)2D (calcitriol) levels.Another reason why the level of 1,25(OH)2D is not useful in assessing vitamin D status is because 1,25(OH)2D is a very short-lived metabolite causing its own destruction by rapidly inducing synthesis of the enzyme 25‑hydroxyvitamin D-24-hydroxylase (Wacker and Holick, 2013).
18b.2.5 Extrarenal production of 1,25‑dihydroxyvitamin D (calcitriol)
The extrarenal pathway of 1,25(OH)2D (calcitriol) is locally produced in almost every tissue in the body (Norman, 2008). As 1,25(OH)2D acts locally, this synthesis pathway is called Paracrine / Autocrine. Activity of extra-renal 25(OH)D-1-α-hydroxylase is not regulated by the hormones that control renal 25(OH)D-1-α-hydroxylase (i.e., PTH and FGF-23). The activity of the enzyme must be induced in the cell. The diverse actions of 1,25(OH)2D, when acting locally as a transcription factor in many different cell types, are called “non-calcemic” or “non-skeletal” and include immuno-modulatory and cell-differentiating properties. It is these properties that have led researchers to investigate vitamin D and its derivatives in the pathogenesis of cancer, respiratory diseases, and immune responses. For further details of these noncalcemic functions see (Norman, 2008) and (Wacker and Holick, 2013).18b.2.6 Serum 25(OH)D
Serum 25(OH)D is a biomarker of vitamin D exposure and status. Both metabolites 25(OH)D and 1,25(OH)2D circulate in plasma. The former, 25(OH)D, reflects the sum of vitamin D from dietary intake and sunlight exposure, whereas plasma 1,25(OH)2D concentrations reflect the immediate physiological need and are under homeostatic control in the kidney. Concentrations of 1,25(OH)2D in plasma are about 0.1% of those of 25(OH)D. In vitamin D deficiency, serum 1,25(OH)2D levels may be normal or even elevated, as a result of increased renal production of 1,25(OH)2D in response to the rise in serum parathyroid levels (Wacker and Holick, 2013). In contrast, plasma 25(OH)D concentrations remain low until a reserve accumulates. As a result, the plasma 25(OH)D concentration reflects medium to long-term vitamin D availability from both dietary and endogenous sources, thus making it the best biomarker of vitamin D exposure and status.18b.3 Vitamin D deficiency in humans
Osteomalacia may occur in severely vitamin D deficient adults, a condition characterized by a failure in the mineralization of the organic matrix of bone. This results in weak bones, diffuse skeletal bone tenderness, proximal muscle weakness, and an increased frequency of fractures. Such disturbances are associated with serum 25(OH)D concentrations below 7.5nmol/L (Haddad and Stamp, 1974). After treatment with vitamin D supplements, serum 25(OH)D values rise and radiological lesions heal (Preece et al., 1975). Osteomalacia prevalence may be high globally due to lack of sun exposure, but remains largely undiagnosed due to the need to have radiographic data (Uday and Högler, 2019). It may occur in adults living in the tropics,who have no sun exposure, such as garment factory workers in Bangladesh (Islam et al., 2008). Low dietary intake may also play a role. A study in Germany of mainly white adults who died accidently, found the prevalence of osteomalacia to be at least 25% based on osteoid volume/bone volume ratio (Priemel et al., 2010).Some adult patients with chronic renal failure, gastrectomy, intestinal malabsorption and steatorrhea arising from celiac disease, inflammatory bowel disease, pancreatic insufficiency, or massive bowel resection, may also develop osteomalacia. Functional disturbances have been described in adults with low serum 25(OH)D concentrations. These include secondary hyperparathyroidism, an increased bone turnover, and reduced bone mass (Chapuy et al., 1997). In the elderly, suboptimal vitamin D status decreases absorption of calcium, a factor associated with a lowering of the bone mineral content during postmenopausal aging.
Rickets occurs in infants and children with severe vitamin D deficiency. In rickets, abnormal softness of the skull (craniotabes) occurs. This may be accompanied by enlargement of the epiphyses of the long bones and of the costochondral junction (rachitic rosary). Bowlegs and knock knees may arise from these bone deformities. Rickets, arising from primary vitamin D deficiency may occur in infants in industrialized countries who are breast-fed without vitamin D supplementation. A supplement of 400 IU (10µg) per day to prevent rickets is often recommended for all infants from birth to 12mo of age, independent of their mode of feeding (Wagner and Greer, 2008; Munns et al., 2016). However, for breastfed infants whose mothers have an adequate vitamin D status, the content of vitamin D in breastmilk can be sufficient because the vitamin D content in breastmilk is dependent on maternal status (Stoutjesdijk et al., 2017). Nutritional rickets can occur in older children, particularly during the adolescent growth spurt (Beck-Nielsen et al., 2009; Uday and Högler, 2019). However, some nutritional rickets is also caused by a lack of calcium, so both vitamin D and calcium should be monitored (Munns et al., 2016; Uday and Högler, 2019).
Metabolic defects also cause rickets, including both vitamin D-resistant rickets (familial hypophosphatemia) and vitamin D-dependent rickets (VDDR type 1). The latter condition is a deficiency of the 25(OH)2D-1-hydroxylase enzyme, while vitamin D-resistant rickets is a defect in proximal renal tubular resorption of phosphate. The yearly incidence of hypophosphatemic rickets in infants 0–0.9y is about 3.9 per 100,000: vitamin D-resistant rickets (VDDR type 1) is very rare (Beck-Nielsen et al., 2009).
18b.4 Food sources and dietary intakes
Natural food sources rich in vitamin D are restricted to fatty fish, organ meats, and UV-exposed mushrooms. In some countries — for example, the United States, Canada, and Finland — fluid milk is fortified with vitamin D. In the United Kingdom and Europe, low amounts of vitamin D can be added to some breakfast cereals, margarine, fat spreads, and vegetable oils, breakfast beverages, and breads (Calvo et al., 2005). In low and medium income countries, fortification of wheat, edible plant-based oil or milk, could reduce vitamin D deficiencies (Cashman and O’Dea, 2019).Intakes from national nutrition surveys provide data on vitamin D in different countries. They show low (3.0µg/d) vitamin D intakes on average in countries where there is little or no vitamin D fortification, such as the U.K. However in countries such as Japan, fatty fish may be consumed in sufficient quantities to provide vitamin D intakes > 7µg/d (Calvo et al., 2005). Canada, where there is mandatory fortification of milk and margarine, has an average intake of 5µg/d which does not meet the adult US/Canadian Estimated Average Requirement (EAR) of 10µg/d. The Canadian government will double the level of mandatory fortification by 2023 to try to reduce the prevalence of inadequacy (Vatanparast et al., 2020).
High Intakes following self-dosing with excessive amounts of vitamin D supplements have been described, although the dose required to induce vitamin D toxicity is uncertain (Hathcock et al., 2007). Vitamin D intoxication does not arise from the consumption of conventional foods (including fortified foods), nor does excess exposure to UVB through sun or artificial lamps cause toxicity (Marcinowska-Suchowierska et al., 2019). Cases of toxicity have arisen, however, from accidental overfortification of milk with vitamin D3, from uncontrolled use of vitamin D mega-doses, and from inappropriate use of vitamin D metabolites. An increased risk for vitamin D toxicity is also associated with certain diseases, including sarcoidosis, tuberculosis, and genetic disorders of rare polymorphisms of enzymes involved in vitamin D metabolism (e.g., idiopathic infantile hypercalcemia) (Wacker and Holick, 2013). Signs of vitamin D toxicity include hypercalcemia (i.e., elevated serum calcium concentrations) and hypercalciuria (elevated urine calcium levels). Hypercalcemia arises from hyperabsorption of intestinal calcium and, to a lesser degree, from the release of calcium from bone and can lead to calcification of soft tissues such as arteries (arteriosclerosis) and the kidney (nephrocalcinosis). The hypercalciuria associated with vitamin D toxicity reflects the presence of excess calcium in the serum arising from the release of calcium from bone.
18b.5 Nutrient reference values for vitamin D
Unlike other essential nutrients, measurements of vitamin D intake using traditional dietary assessment methods cannot be used to measure dietary exposure because some of the requirement for vitamin D can be met from skin synthesis, as noted earlier. Instead a surrogate biomarker is used to measure exposure to vitamin D; serum 25 hydroxyvitamin D (25(OH)D) is the biomarker of choice. Serum 25(OH)D measures exposure to vitamin D from the effects of both diet and sunlight as noted earlier, and associations between concentrations of serum 25(OH)D and functional biomarkers of bone health have been considered when setting Nutrient Reference Values (NRVs) for vitamin D by several agencies. The evidence for extraskeletal outcomes has been considered inadequate, inconsistent, or insufficient to develop Nutrient Reference Values (NRVs). Nevertheless, in view of the variation in sunlight exposure and the variable response to that exposure, as well as concerns about skin cancer, several agencies, including the IOM (2011), EFSA (2016), and the UK (2016) have set their NRVs based on the assumption of minimal or no sunlight exposure. This means that in the presence of cutaneous vitamin D synthesis, the requirement for dietary vitamin D may be lower than that set, or may even be zero.The US IOM (2011), as an example, set an Estimated Average requirement (EAR), the requirement to satisfy the need of half the population, of 10µg/d for all children and adults age 1–70y, as necessary to maintain bone health and achieve a serum 25(OH)D concentration of 40nmol/L (16ng/mL). The Recommended Dietary Allowance (RDA), designed to cover the requirements for 97.5% of the population, was set at 15µg/d, a level that corresponded to a serum 25(OH)D concentration of 50nmol/g (20ng/mL). A higher RDA level (i.e., 20µg/d) was recommended for those over 70y, due to age-related inefficiencies in vitamin D metabolism. For infants 0–12months, IOM set an Adequate Intake (AI) of 10µg/d because there was not enough information to establish an EAR for this group.
Several other countries and regions have also restricted their recommendation to a single level — the AI (or equivalent) — because of insufficient evidence, including Australia and New Zealand (NHMRC, 2006), the European Union (EFSA, 2016), and most recently, the United Kingdom (SACN, 2016), although here only for infants and children less than 4y. However, the target serum 25(OH)D concentrations on which the AIs are based vary, ranging from at least 25nmol/L (10ng/mL) in Australia and New Zealand (NHMRC, 2006), and the UK (SACN, 2016), to 50nmol/L (20ng/mL) in the EU (EFSA, 2016). As a consequence, the AI levels range from about 10µg/d to 15µg/d for most age groups, similar to those for several other European countries (Spiro and Buttriss, 2014).
The UK , like the US has also set a Recommended Nutrient Intake (equivalent to the RDA) for all life-stage groups (including pregnant women, lactating women, and the elderly), except those < 4y. However, for the UK (SACN, 2016), the RNI is 10µg/d in contrast to the 15µg/d RDA set by IOM (2011). No EAR has been set by the UK. Cashman et al. (2008), have emphasized that dietary levels of about 10–15µg/d may not be adequate to keep most of the adult population in Europe above a higher target value of 50nmol/L in winter without adequate sun exposure in the summer season.`
Several agencies have established a Tolerable Upper Intake Level (UL) for vitamin D to discourage potentially dangerous self-medication. The UL is defined as the highest average intake that is likely to pose no risk. For infants 0–6mo, the IOM set an UL of 25µg/d, rising with age through childhood to 100µg/d for persons aged 9y and older. This upper limit corresponds to an average serum total 25(OH)D levels of 125nmol/L (50ng/mL) (2011).
The UL values for the European Food Safety Authority (EFSA) start for infants aged 7–11mo with an UL of 35µg/d which rises to 100µg/d for persons aged > 11y (EFSA, 2016). Data on the long-term adverse effects of high doses of vitamin D are limited, however, and caution is necessary when setting an UL for chronic intake (Aloia, 2011).
The Endocrine Society, focusing on clinical use for patients at high risk of deficiency with rickets, osteomalacia, osteoporosis, chronic kidney disease, etc., and some special populations (e.g., pregnant women) , have set a higher adult UL of 250µg/d (Holick et al., 2011) , a recommendation that is not appropriate for healthy individuals in the population. This distinction is important, and if not recognized, could result in inappropriate dietary recommendations for healthy individuals, for whom the NRVs should be used (Aloia, 2011).
18b.6 Biomarkers of vitamin D status
Historically, vitamin D status was assessed indirectly by measuring alkaline phosphatase activity, as well as calcium and phosphorus concentrations in serum: all very nonspecific indices. Methods are now available for the direct measurement of vitamin D metabolites in serum, and these are described below. If possible, these measurements should be performed in conjunction with an assay of serum parathyroid hormone and some functional assessment of skeletal health. In adults, this assessment may include measurement of bone mineral content or bone mineral density. In children, in extreme cases of rickets, bony deformities such as enlarged fontanelle, rachitic rosary, and swollen joints are clinical signs of rickets, whereas knock knees or bowed legs are clinical signs of the associated osteomalacia noted in growing children (Uday and Högler, 2019).18b.6.1 Serum 25‑hydroxyvitamin D
Serum 25‑hydroxyvitamin D is the circulating metabolite of vitamin D that is the most abundant and has the longest half-life of all the vitamin D derivatives. Concentrations of 25(OH)D in serum (or plasma) are also the most useful measure of vitamin D exposure and status in humans, as they reflect the total supply of vitamin D from both cutaneous synthesis and dietary intake of either vitamin D2 or vitamin D3 (Wacker and Holick, 2013). Moreover, they can be used to define vitamin D deficiency, insufficiency, hypovitaminosis, sufficiency, and toxicity (IOM, 2011). Concentrations in healthy adults vary from 30–130nmol/L, depending in part on exposure to solar ultraviolet light.18b.6.2 Factors affecting serum 25 hydroxyvitamin D
Seasonal and latitude effects on serum 25 hydroxyvitamin D status are marked in many areas of the world. Subjects living north of latitude 33°N and south of latitude 33°S (Wacker and Holick, 2013) have reduced dermal vitamin D synthesis during winter, and show the highest serum 25(OH)D levels in the late summer, and the lowest in late winter. In a U.K. national survey of people > 65y (Finch et al., 1998), mean plasma 25(OH)D concentrations were significantly higher in free-living participants surveyed in the summer (July–September) than in the winter (Figure 18b.3).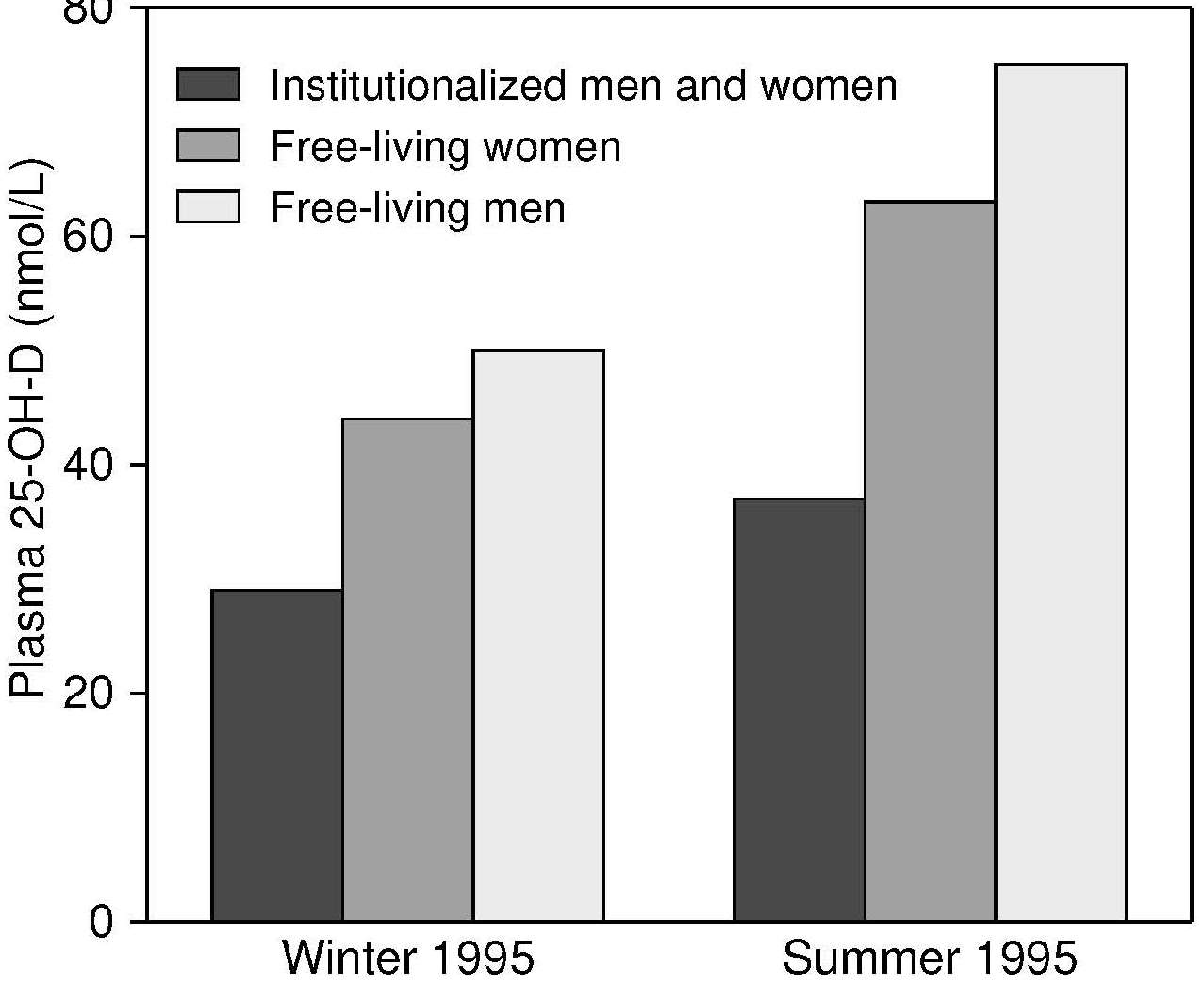
Only UVB rays (290–315nm) elicit synthesis of vitamin D3 (cholecalciferol). When the incident angle of the sun is low, UVB does not reach the earth (Grant et al., 2016). The “Shadow Rule” states that if one's height is longer than the length of one's shadow, vitamin D synthesis is possible; if not, the sun's incident angle is too low to provide UVB. This also explains why the best time for vitamin D synthesis is between 10:00 and 14:00 in summer in temperate countries or year-round in low latitude regions. Other factors affecting dermal synthesis are described below.
Age-related changes from infancy to adulthood in the concentrations of serum 25(OH)D can be marked. Newborn infants have serum concentrations that correlate with maternal 25(OH)D concentrations. Breastfed infants, as noted above, are at risk of poor vitamin D status unless mothers have an adequate vitamin D status and can pass on metabolites in their milk (Stoutjesdijk et al., 2017). Vitamin D metabolites are lower when measured in cord blood. Serum 25(OH)D levels in children of both sexes decline with increasing age ( Gregory et al., 2000), a trend that may reflect both behavioral factors (diet and sun exposure) as well as biological effects (increasing requirements of vitamin D with growth).
Studies indicate that calcium absorption by premature infants is not only vitamin D dependent; however, concern remains about the lack of data to outline the vitamin D needs for premature infants (Taylor et al., 2019).
Older adults are particularly vulnerable to low levels of serum 25(OH)D (McKenna et al., 1985). Lifestyle factors that reduce vitamin D status include low dietary intakes of vitamin D and limited sun exposure with a greater use of sunscreens and umbrellas. In addition, there are biological reasons for the low status including a reduced capacity of the skin to produce vitamin D resulting from a reduction of 7-dehydrocholesterol in the skin, and impaired intestinal absorption of ingested vitamin D (Wacker and Holick, 2013). In countries such as Canada where low vitamin D status is related to osteoporosis, it is recommended that older adults take vitamin D supplements and age-related declines in vitamin D status as measured by serum 25(OH)D have not been observed in Canadian national survey data (Brooks et al., 2017).
Sex and Gender differences in concentrations of serum 25(OH)D have been noted, although no consistent pattern has emerged from national survey data in the USA (Wacker and Holick, 2013) and Canada (Brooks et al., 2017). Differentiating biological (sex) effects from lifestyle effects (gender)such as differences in food preferences, clothing, and amounts of sun exposure during work or leisure may be difficult. In some countries, consumption of fortified foods and supplements as well as sunscreen use and sun avoidance practices, differ between males and females of all ages.
Skin pigmentation differences, often seen when comparing ethnic or racial groups, greatly influences serum 25(OH)D concentrations. Skin pigmentation reflects the amount of the pigment melanin that absorbs UVB rays and lowers the amount of UVB acting on previtamin D. In the USA, for example, serum 25(OH)D concentrations in African-Americans and Hispanics are much lower than in non-Hispanic whites (2011). In European countries, African immigrants now living in northern latitudes have a greater risk of vitamin D deficiency compared to non-migrants. As an example, of the patients with nutritional rickets in Denmark, 74% were immigrant children (Beck-Nielsen et al., 2009). Not all differences in vitamin D status between ethnic groups are due to skin pigmentation. Behavioral differences such as dietary intakes, clothing preferences, and sun avoidance practices are also important factors.
Melanin in skin does not block all cholecalciferol synthesis, but it is slowed. Webb and Engelsen (2006) have calculated the time needed for a person with each of the different Fitzpatrick categories of skin type to burn with sun exposure. Also shown in Table 18b.1,Fitzpatrick Skin Type |
How skin responds to sun exposure |
Minutes to make 25µg (1000IU) |
---|---|---|
I | Always burn never tan | 4 |
II | Burn slightly then tan slightly | 6 |
III | Rarely burn tan moderatelu | 7 |
IV | Never burn, tan moderately e.g. Mediterranean | 10 |
V | Never burn, tan darkly Asian, Indigenous American, Pacific Islander | 13 |
VI | Never burn, tan very darkly; Australian Aborigine, Tamil, West African | 21 |
Sunscreen lotions are used to deliberately block UV rays reaching the skin. Sunscreens are labeled with a sun protection factor (SPF) number which indicates the amount of UV blocked. An SPF blocks at 1/SPF, so that a product having an SPF of 8 would allow only 1/8 (12.5%) of the UV to penetrate the lotion and reach the skin. Theoretically sunscreens should reduce vitamin D synthesis. In a national survey in Canada, however, participants answering “yes” to using sunscreen had higher 25(OH)D levels (2.4 ±1.1nmol/L; P < 0.001) (Brooks et al., 2017). Several reasons may account for this seemingly abherrant finding. Users may apply sunscreens poorly or incompletely (for example answer “yes” to use but only apply to face). Alternatively, sunscreen users may spend more time outdoors. Other behavioral differences may exist, for example in the Canadian survey, sunscreen users were more likely to take vitamin D supplements than nonusers.
Smoking is associated with lower serum 25(OH)D concentrations. This may partly explain the reported increased risk of osteoporosis among smokers. The mechanism is unclear, but the relationship does not appear to result exclusively from additional confounding lifestyle factors (Brot et al., 1999). In a large study of adults in Australia, both male and female nonsmokers, including ex-smokers, had higher mean levels of serum 25(OH)D compared to current smokers (Gill et al., 2017).
Obesity, prevalent in many population groups worldwide, is associated with a trend towards lower serum 25(OH)D levels (Brooks et al., 2017; Wacker and Holick, 2013). Biologically, this trend can be attributed to vitamin D, whether from cutaneous or dietary sources, being deposited in adipose tissue, where it is not bioavailable (Wacker and Holick, 2013). Some researchers have found that obesity in men has less of an effect on reducing 25(OH)D than in women, perhaps because of gender differences in behavioral aspects such as sun avoidance (Rockell et al., 2006). Nevertheless, body weight must be considered when evaluating vitamin D status
Disease conditions affecting the gastrointestinal tract, the liver and kidneys may cause a secondary deficiency of vitamin D (Table 18b.2).Causes of Secondary vitamin D Deficiency | |
---|---|
Pathology | Diseases |
Malabsorption of fat reduces absorption of dietary vitamin D |
Cystic fibrosis Celiac disease, Whipple's disease, Crohn's disease, Bypass surgery. |
Liver failure prevents production of 25(OH)D |
Cirrhosis Hepatitis |
Inability to produce 1,25(OH)2D in kidney | Chronic kidney disease |
Medications | |
Drugs reducing Vitamin D absorption |
Cholesterol-lowering agents: cholestyramine Weight loss drug orlistat and food additive olestra |
Drugs reducing 25(OH)D levels due to increased catabolism |
Anticonvulsant medications such as carbamazepine, phenobarbital, and phenytoin, gabapentin. Antiretrovirals agents such as ritonavir and efavirenz, valproic acid (AIDS treatment) Histamine H2 receptor antagonist cimetidine |
Drugs Impairing vitamin D metabolism |
Oral corticosteroids such as glucocorticoids |
Medication use can affect vitamin D status. Any drug which affects liver or kidney cytochrome enzymes will likely affect conversions of vitamin D metabolites. Table 18b.2 provides a list of drugs known to impact vitamin D status. While this list does not cover all possible secondary causes of deficiency, it emphasizes the need to monitor disease states and medication use as possible reasons for vitamin D deficiency. As is described below, the requirement for vitamin D may be elevated in persons who have chronic conditions or for whom medication use is required.
Magnesium status may impact 25(OH)D levels and therefore vitamin D status through the requirement for two enzymes of vitamin D metabolism: 25(OH)D-1-α-hydroxylase and 25(OH)D-24-hydroxylase. In magnesium deficiency, there is a reduction in the active form 1,25(OH)2D associated with “Mg-dependent vitamin-D-resistant rickets” (Dai et al., 2017). More research is needed to determine the intake of magnesium that affects vitamin D status.
Analytical methods have a marked effect on serum 25(OH)D concentrations. To overcome inter-assay differences, and establish the accuracy and precision of the assay, verified standards should be run with every batch using the Vitamin D Standardization Program. See Section 18b.9.
18b.8 Interpretive criteria
Table 18b.3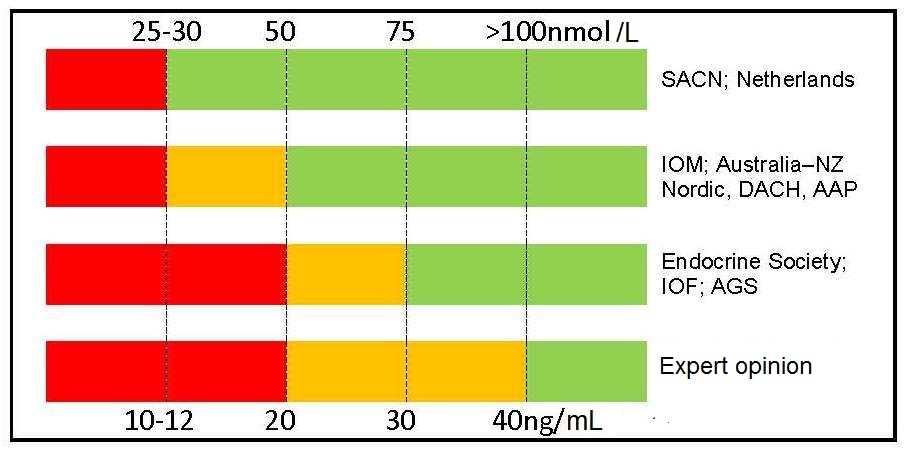
Agreement exists Table 18b.3 between two groups of nations (i.e., United Kingdom, the Netherlands with Australia/New Zealand, European Union, USA) for the cutoff point to define severe deficiency (i.e., 25–30nmol/L, 10–12ng/mL), but there is much less agreement for cutoff points to define vitamin D insufficiency, vitamin D sufficiency, and vitamin D toxicity. Conflicting recommendations have been compiled by some non-governmental medical societies and organizations.
In 2011, the US Institute of Medicine (IOM) published cut-off points for serum total 25(OH)D that represent public health guidelines for generally healthy non-diseased populations Table 18b.4Interpretation | IOM (ng/mL) |
Endocine Society (ng/mL) |
---|---|---|
Deficient | <12 | <20 |
Insufficient | 12–20 | 21–29 |
Sufficient | 20–30 | 30–100 |
No added benefit | 30–50 | |
Possible harm | >50 | >100 |
Note the levels set by IOM differ from those set for clinical practice by the Endocrine Society in 2011, and presented in Table 18b.4 (Holick et al., 2011). However, these are intended as guidance for clinicians for the evaluation, treatment, and prevention of vitamin D deficiency, with emphasis on the care of patients who are at high risk of deficiency (e.g., those with rickets, osteomalacia, osteoporosis, chronic kidney disease, etc). Such practice guidelines to treat disease should not be applied to the apparently healthy population. Clearly, more research is urgently required using assays that meet the standardized criteria developed by the Vitamin D Standardization Program (VDSP) (Section 18b.9) to develop a consensus on a single set of rigorous interpretive criteria for serum total 25(OH)D levels relative to vitamin D status and interventions that are appropriate for both public health and clinical needs. In the meantime, readers are advised to use the IOM (2011) cutoffs proposed and listed in Table 18b.4 for public health use.
18b.9 Measurement of serum 25‑hydroxyvitamin D
There are three main methods for measuring serum 25(OH)D: immunoassay, high performance liquid chromatography (HPLC), and liquid chromatography with tandem mass spectrometry (LC-MS/MS) However, there are two main steps that must be undertaken before selecting a method for assaying total 25(OH)D in serum. The first step is verification of “fit-for-purpose” . This step is only necessary when an immunoassay is the intended method and is necessary because some immunoassays are not appropriate for certain patient population groups (e.g., those with certain disease states, pregnant women, and vegetarians such as vegans) (Sempos and Binkley, 2020). In addition, the chosen immunoassay should have an appropriate measurement range for the intended study population (e.g., measure 25(OH)D levels in persons who are deficient). The “fit for purpose” step can be accomplished by testing the immunoassay against a Vitamin D Standardization Program (VDSP) standardized LC-MS/MS assay.The second step required is standardization, a process whereby the intended assay is calibrated to meet the VDSP performance criteria (i.e., total coefficient of variation (CV) < 10% and a mean bias with the range of –5% to +5%). Some assays, even when based on a similar methodology, are less accurate and precise, making comparison of data across assays or laboratories difficult. The gold standard analytical method is LC-MS/MS and that should always be the method of choice for national surveys. However, even the LC-MS/MS method must meet the assay standardization criteria of the VDSP. Serum total 25(OH)D measurements can be 'prospectively' standardized or 'retrospectively' standardized, using methods developed by VDSP; see Sempos et al., 2017 and Durazo-Arivizu et al., 2017 for more details. A standardized laboratory measurement is defined as one that provides the 'true' serum total serum 25(OH)D concentrations. Several NIST Standard Reference Materials — SRM 972a, 2973, and 1949 — which provide target values for 25(OH)D2 and 25(OH)D3 are available to check on the accuracy and precision of the chosen assay. NIST SRM 1949 also provides target values for serum total 25(OH)D values for the first, second, and third trimester of pregnancy and these should be used in studies of pregnancy.
18b.10 Measurement of serum 1,25‑dihyroxyvitamin D
The active form of vitamin D is 1,25(OH)2D (also called calcitriol) interacts with its nuclear receptor in the intestine, bone, and kidney for most of its functions to regulate calcium and bone metabolism. 1,25(OH)2D also has many other noncalcemic cellular actions that reside in the Paracrine/Autocrine pathway (Norman, 2008; Wacker and Holick, 2013).
1,25(OH)2D in serum is not a useful marker of vitamin D status because it has a short half-life (4–6h) and levels are under stringent homeostatic regulation by factors at the site of 1,25(OH)2D synthesis in the kidney. Hence, it is not surprising that no seasonal variation in serum 1,25(OH)2D concentrations has been reported (Landin-Wilhelmsen et al.,1995).
Synthesis of 1,25(OH)2D in the kidney is stimulated by low serum concentrations of calcium or phosphorus and is inhibited by excess 1,25(OH)2D. In cases of vitamin D sufficiency, a positive relationship exists between serum 1,25(OH)2D and 25(OH)D concentrations (Need et al., 2000), presumably because 25(OH)D is the substrate for 1,25(OH)2D. In vitamin D deficiency, however, this relationship is reversed because with a fall in serum 25(OH)D concentrations there is a rise in the concentration of parathyroid hormone resulting in an increase in the renal production of 1,25(OH)2D ( Figure 18b.2) (Wacker and Holick, 2013). As a result, the circulating concentrations of 1,25(OH)2D often become normal or even elevated. In contrast, serum 1,25(OH)2D levels decrease in renal disease (which affects the enzyme, 25(OH)D-1-α-hydroxylase); levels are very low in anephric patients (i.e., those lacking a functional kidney), and in patients on hemodialysis. Even in normal healthy subjects, concentrations of serum 1,25(OH)2D are in the picomolar range, making analysis difficult (Zittermann, 2003). Serum 1,25(OH)2D concentrations ranging from 60–100pmol/L are normal in adults; concentrations in children tend to be higher. Methods of analysis include HPLC, LC-MS/MS and radioimmunoassay. (Zittermann et al., 2016).
18b.11 Other vitamin D metabolites
The 24 hydroxy metabolites 24,25‑dihydroxyvitamin D and 1,24,25‑trihydoxyvitamin D can be measured to assess the conversion of 25(OH)D and 1,25(OH)2D to their respective inactive metabolites. Recently, measurement of metabolite-to-parent compound ratios were used to estimate metabolite hydroxylase activity in a study comparing vitamin D2 and vitamin D3 bolus doses (Martineau et al., 2019). In the future, additional vitamin D metabolites will be measured as the vitamin D field continues to expand. For example, measurement of 3-epi-25(OH)D3 and vitamin D-binding protein (VDBP) may help in understanding aspects of vitamin D status (Sempos and Binkley, 2020). Hence, investigators should store serum samples appropriately for possible future analyses using LC-MS/MS together with the available reference materials for standardization.
18b.12 Serum alkaline phosphatase
Alkaline phosphatase (EC 3.1.1.1) activity in serum can be used as an indirect measure of vitamin D status. Activity increases in osteomalacia in adults and childhood rickets but is generally normal in osteoporosis. Increases in the activity of alkaline phosphatase are usually proportional to the severity of vitamin D depletion. For example, elderly Irish individuals with serum 25(OH)D levels indicative of severe or marginal vitamin D depletion had slightly higher serum alkaline phosphatase activity than those with 25(OH)D levels classified as replete (Figure 18b.4).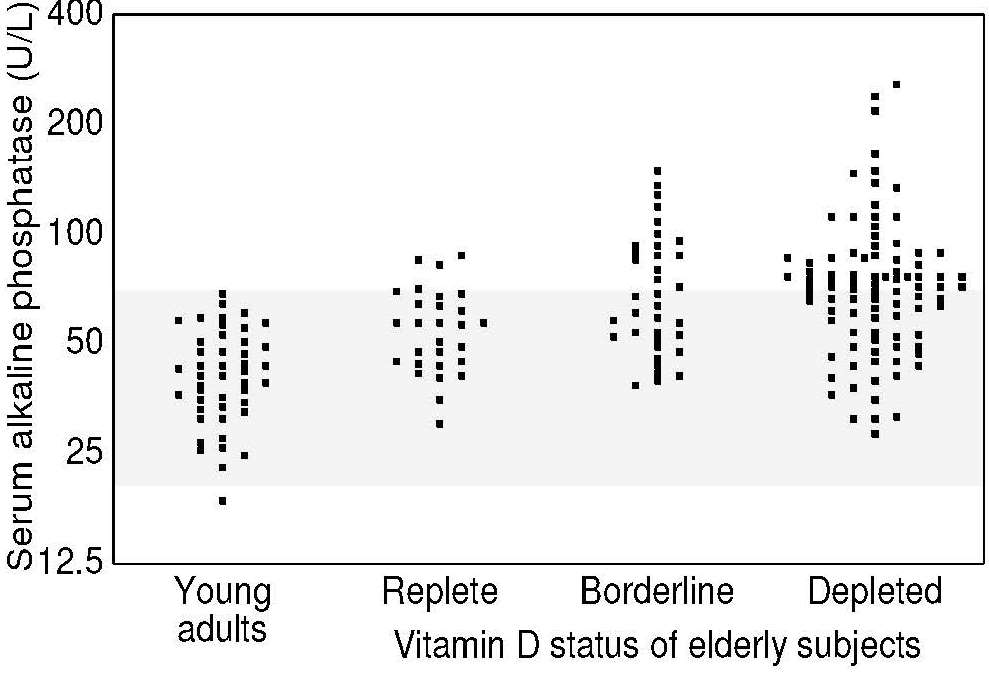
Serum alkaline phosphatase activity is also affected by sex and age, again in the opposite direction to changes in the levels of serum 25(OH)D. Serum alkaline phosphatase activity is significantly higher in females relative to males and in older versus younger adults; activity is also higher in growing children and pregnant women, especially during the third trimester (McKenna, 1992). In older surveys such as the U.K. national survey of young people 4–18y, mean alkaline phosphatase activity was measured. The lowest level was found in the oldest adolescents (15–18y), especially among the girls (Gregory et al., 2000).
The activity of serum alkaline phosphatase is also altered by various disease states such as hyperparathyroidism, Paget's disease, secondary bone cancer, and cholestasis (Sauberlich, 1999). Serum alkaline phosphatase activity may decrease in zinc deficiency but, as noted earlier, appears to be close to normal in osteoporosis in contrast to osteomalacia where it is high (Uday and Högler, 2019).
In general, measurement of alkaline phosphatase activity in serum is best used to confirm a clinical diagnosis of vitamin D deficiency, or as a screening tool, but it is not very useful for detecting subclinical vitamin D deficiency. The diagnosis of osteomalacia can be made in the presence of high alkaline phosphatase activity accompanied by high PTH, low dietary calcium intake (< 300mg/d) and/or low serum 25(OH)D (< 30nmol/L) (Uday and Högler, 2019).
18b.12.1 Interpretive criteria
Serum alkaline phosphatase activity is normally expressed as U/L. The reference range for normal adults is 30–135U/L (Gregory et al., 2000). Total plasma alkaline phosphatase activity was measured in the past U.K. national surveys (Gregory et al., 2000, Finch et al., 1998) , with the exception of the survey on pre-school children. Mean, median, and lower and upper 2.5 or 5th percentiles by age and sex are presented.
18b.12.2 Measurement of alkaline phosphatase
Several methods are available for the assay of serum or plasma alkaline phosphatase (ALP); for plasma, heparinized blood samples should be used (Bessey et al., 1946). Total alkaline phosphatase measures all sources of enzyme activity including liver, while bone-specific alkaline phosphatase (BALP) measures activity derived from osteoblast activity. Higher total or bone alkaline phosphatase levels accompanied by low urinary calcium could prompt investigation of vitamin D deficiency (Kennel et al., 2010). The assay of total alkaline phosphatase activity is sufficient evidence as long as other liver enzymes are normal. The activity of serum or plasma alkaline phosphatase should be expressed as U/L. The within-subject coefficient of variation for serum/plasma alkaline phosphatase activity ranges from 4.6% to 9.2%, depending on the time frame and dietary regimen (Gallagher et al., 1989). The enzyme is reasonably stable in frozen serum or plasma.
18b.13 Serum parathyroid hormone
Parathyroid hormone (PTH) levels in serum or plasma are considered to be a functional biomarker of vitamin D status in the normocalcemic state. In vitamin D deficiency, when calcium absorption is reduced, serum PTH levels rise to induce calcium mobilization from the bone and increase tubular reabsorption of calcium in the kidney. In this way, serum calcium is maintained at a physiologically optimum level. As a result, serum PTH concentrations are inversely related to serum 25(OH)D levels (Chapuy et al., 1997; Need et al., 2000; Wacker and Holick, 2013).Threshold values for serum 25(OH)D concentrations that induce an increase in serum parathyroid secretion vary. In a study of Australian postmenopausal women, the threshold value was 40nmol/L (Need et al., 2000), whereas in French adults it was 78nmol/L (Chapuy et al., 1997). In the 2011 IOM report, studies examined showed that PTH is inversely associated with serum 25(OH)D concentrations at lower 25(OH)D concentrations but there was inconsistent evidence for a threshold above 27nmol/L. Such variable evidence for a threshold may result from different assays being used, both to measure serum PTH and serum 25(OH)D. Even small increases in PTH levels may have a negative influence on bone mass and increase the risk of non-vertebral fracture (Chapuy et al., 1997). Hence, a combination of serum PTH and serum 25(OH)D concentrations is often recommended as an indicator of vitamin D status.
Serum PTH levels increase with age, independent of 25(OH)D, ionized calcium, phosphate, and renal function (Carrivick et al., 2015 ). Further research is required to explore the underlying mechanisms and clinical relevance. Season also influences PTH concentrations, as might be expected from seasonal variations in 25(OH)D. Parathyroid hormone can be measured by radioimmunoassay using commercial kits. (Zittermann et al., 2016).
18b.14 Calcium and phosphorus in serum and urine
Many studies of vitamin D status have included the measurement of calcium and phosphorus concentrations in serum or urine. The measurements are most useful if combined with the measurements of serum 25(OH)D and PTH concentrations. In vitamin D deficiency in infants and children, the serum calcium and phosphorus levels are usually reduced. For example, significantly lower mean serum calcium concentrations were reported in French neonates with serum 25(OH)D concentrations < 30nmol/L and elevated PTH concentrations, in comparison with individuals with normal values for these biochemical parameters (Zeghoud et al., 1997). Unlike total serum calcium alone, the existence of such a triad of biochemical disturbances strongly indicates vitamin D deficiency.
Serum calcium is also used to identify possible vitamin D intoxication. In such cases, concentrations of serum 25(OH)D and serum calcium are elevated and provide additional evidence for hypervitaminosis D.
The response of urinary calcium and phosphorus levels to changes in vitamin D status varies, as excretion of these minerals is also affected by dietary intakes. Early osteomalacia can be detected as a decrease in urinary calcium to creatinine ratio (Uday and Högler, 2019). Changes in urinary calcium and phosphorus concentrations, however, are not specific for vitamin D status. Details of the measurement of calcium and phosphorus in serum and urine are given in Chapter 23.